Chapter 3: New Line Development and New Line Evaluation: Single-cross Hybrid
Rita H. Mumm
In Chapter 2, the different concepts and processes, and pertinent considerations in the choice of parents, creation of progeny and materials for testing, and evaluation and selection of desirable lines in cultivar development were examined using a pure line variety of a self-pollinated crop, soybean. In this chapter, we will examine the changes to the process and the product pipeline that would be needed to develop improved hybrid cultivars. The example cited is a single-cross maize hybrid characterized by complete crop uniformity (Fig. 1).
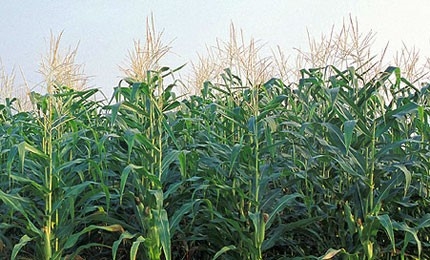
Hybrid Vigor = Heterosis
Hybrid cultivars exploit the advantage of heterosis, also referred to as hybrid vigor. The performance of the hybrid is superior to either parent of the hybrid (Fig. 2).
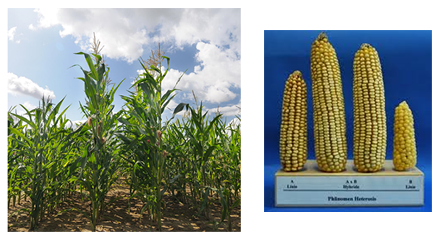
Heterosis Quantified
Heterosis can be quantified for any given cross and it is typically expressed as a percentage:
[latex]\dfrac{100(\text{hybrid - MP})}{\text{MP}}[/latex]
where MP is the mid-parent mean (i.e. mean of the parents’ performance).
For example: if MP = (5.0 + 4.4) / 2 = 4.7 t/ha and hybrid yield is 11.5 t/ha, then mid-parent heterosis =
[latex]\dfrac{100(11.5-4.7)}{4.7}=145\%[/latex]
Heterosis can also be quantified for any given cross in terms of performance of the better parent:
[latex]\dfrac{100(\text{hybrid - BP})}{\text{BP}}[/latex]
where BP is the mean of the better parent (i.e. max [P1, P2]).
For the case above, better parent (also called “high parent”) heterosis =
[latex]\dfrac{100(11.5-5.0)}{5.0}=130\%[/latex]
Heterosis is the Opposite of Inbreeding Depression
The term “heterosis” was coined by George Harrison Shull, who laid the foundation for a more comprehensive understanding of the genetic basis for heterosis based on his work in corn.
He observed that:
- An open-pollinated variety of corn was a complex mixture of hybrids, each plant of a different genotype.
- Inbreeding reduced hybrid vigor; crossing restored it.
- Hybrid vigor is not permanent; hybridization must be done each generation to get the added benefit.
- Inbreds differ in the level of hybrid vigor produced through crossing (combining ability).
- Inbred progeny show more defects than their parents.
George Shull interpreted heterosis as the opposite of inbreeding depression (Shull, 1909). He proposed that the variability among strains undergoing inbreeding, including loss of vigor, was a consequence of segregation and eventual homozygosis of desirable and deleterious alleles. With inbreeding, deleterious alleles are unmasked. And he concluded that heterosis is the product of parent lines compensating for each other. Shull (1908, 1952) applied the concept of heterosis to plant breeding: “increased vigor, size, fruitfulness, speed of development, resistance to disease and insect pests or to climatic rigors manifested by crossbred organisms as compared with corresponding inbreds as the specific result of unlikeness in the constitutions of uniting parental gametes.”
Dominance Driving Heterosis
Eugene Davenport (1908) proposed the Dominance Hypothesis of heterosis. That is, dominant alleles improve fitness of the hybrid by masking deleterious recessive alleles. Edward M. East (1908) and George H. Shull (1908) independently proposed the Overdominance Hypothesis, which declares that certain combinations of alleles represent an advantage in the heterozygote due to over-expression of the gene involved.
Epistatic Gene Action Implicated
Sewall Wright (1922) established that the relationship between mean performance and the decrease in heterozygosity should be linear, regardless of the degree of dominance (i.e., partial, complete, over-dominant) unless linkage or epistasis is involved.
Several studies demonstrated epistasis as a factor in heterosis but accounted for a relatively small portion of the total variability (approximately 10% in Stuber and Moll (1971)).
Dominant gene action is considered the primary factor underpinning heterosis, although more detail on the mechanism is lacking. Additivity is also implicated.
Genetic Diversity Required
The oldest and most famous heterotic pattern is U.S. Yellow Dent corn.
In 1922, Frederick D. Richey observed that hybrids between varieties of different endosperm types in maize resulted in higher yield than varieties of the same endosperm type, suggesting that genetically or geographically distant parents exhibited increased heterosis in hybrid combination. This led to the development of the Reid Yellow Dent x Lancaster Sure Crop pattern, which was the basis for the predominant heterotic pattern developed in U.S. maize: Stiff Staff Synthetic (SSS) x non-Stiff Stalk (NSS).
We can learn a great deal from this important development.
What is a Heterotic Pattern?
A heterotic pattern is a specific pair of heterotic groups whose crosses express high heterosis (and hybrid performance) (Fig. 3). Genetic diversity is necessary but not sufficient.
A heterotic group is a group of related or unrelated individuals from the same or different populations, displaying similar combining abilities when crossed with genotypes from other germplasm groups.
Within a heterotic group, there may be a group(s) of individuals that are more genetically similar to one another than to members of the heterotic group at large. Such a group is referred to as heterotic subgroup.
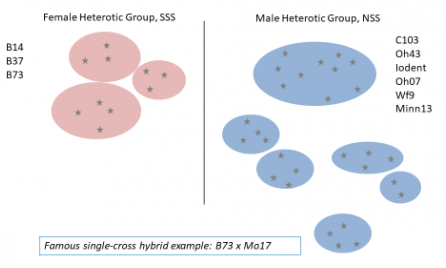
Properties of a Heterotic Pattern
A heterotic pattern has the following characteristics:
-
- Genetic diversity between the two heterotic groups. Diversity is necessary but not sufficient for expression of heterosis. That is, two genetically diverse lines do not necessarily display heterosis in hybrid combination, but two lines that display heterosis in hybrid combination are always genetically diverse.
- General combining ability is expressed between inbreds from opposite heterotic groups.
- Specific combining ability is expressed between specific pairs of inbreds from different heterotic groups… and exploited by plant breeders!
Preserving Genetic Diversity Between Heterotic Groups
The heterotic pattern must be taken into account when choosing parents to create breeding populations. The principles of choosing prospective parents with the best performance for key traits and choosing pairs that represent genetically diverse sources of favorable alleles still hold. However, with hybrid cultivars, it is critical to preserve heterosis between heterotic groups. Therefore, breeding crosses are made within a heterotic group, not between heterotic groups.
Improving a Specific Single-cross Hybrid
Consider a specific single-cross corn hybrid we will call [latex]\text{H}_{Now}[/latex] with parents [latex]\text{I}_1[/latex] (from heterotic group 1) and [latex]\text{I}_2[/latex] (from heterotic group 2).
[latex]\Large\text{I}_1\times \text{I}_2[/latex]
[latex]\Large\downarrow[/latex]
[latex]\Large\text{H}_{Now}[/latex]
Suppose we want to improve this hybrid. How would we go about it?
Improving One Parent of a Specific Hybrid
One of the parents of the hybrid, say [latex]I_1[/latex], could be crossed to a line from the same heterotic group that represents a source of new favorable alleles. Progeny of this breeding cross could be evaluated for their performance in hybrid combination with a line from the opposite heterotic group (called a tester) to make selections (Fig. 4). In this case, a superior progeny advanced to homozygosity would represent a new inbred ([latex]I_{New}[/latex]) which when combined with [latex]I_2[/latex] results in an improved hybrid, [latex]I_{New}[/latex], which outperforms [latex]H_{Now}[/latex].
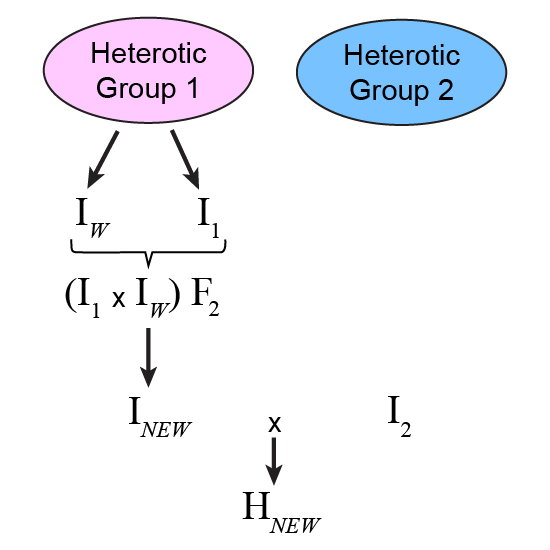
Improving Both Parents of a Specific Hybrid
In practice, breeding crosses are typically made within each heterotic group to improve both sides of the pedigree (Fig. 5). Progeny from each cross are evaluated with respect to performance in hybrid combination with a tester(s) from the opposite heterotic group, especially for traits involving non-additive gene action. The testers can be the parents of [latex]H_{Now}[/latex] or other prominent lines from the opposite heterotic group.
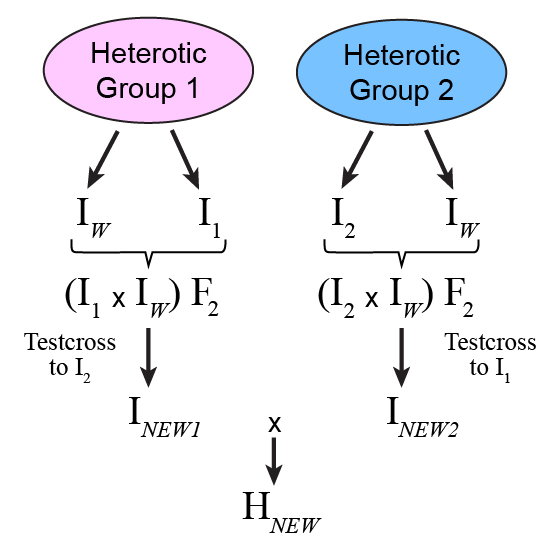
Using a Prospective Source of New Favorable Alleles
Consider a source of favorable alleles for key traits, say [latex]I_W[/latex], to improve [latex]H_{Now}[/latex]:
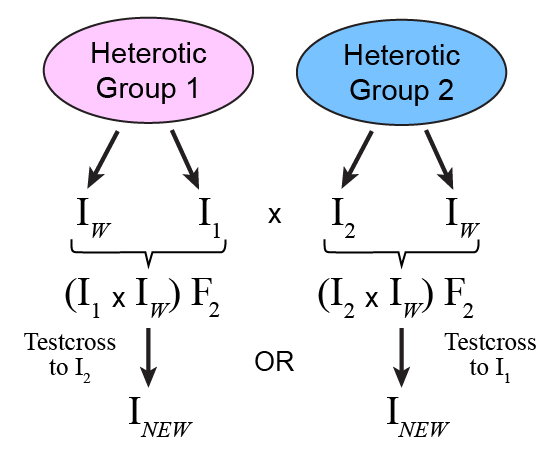
[latex]\text{I}_{W}[/latex] could be crossed to [latex]\text{I}_{1}[/latex] to create [latex]\text{F}_{2}[/latex] families that can be evaluated for their performance in hybrid combination with [latex]\text{I}_{2}[/latex]. A new superior inbred resulting from [latex]\text{I}_{1}[/latex] x (that is, [latex]\text{I}_{New}[/latex] ) could be used in combination with [latex]\text{I}_{2}[/latex] to produce an improved hybrid, [latex]\text{H}_{New}[/latex] (Fig. 6).
Alternatively, [latex]\text{I}_{W}[/latex] could be crossed to [latex]\text{I}_{2}[/latex] to create F2 families that can be evaluated for their performance in hybrid combination with [latex]\text{I}_{1}[/latex] with the recovery of a new superior inbred.
To which inbred ([latex]\text{I}_{1}[/latex] or [latex]\text{I}_{2}[/latex]) should [latex]\text{I}_{W}[/latex] be crossed to create a breeding population that will ultimately contribute to development of a new improved hybrid, [latex]\text{H}_{New}[/latex]?
This answer is easy if you know the pedigree of inbreds [latex]\text{I}_{W}[/latex]and [latex]\text{I}_{1}[/latex] and [latex]\text{I}_{2}[/latex]. You would cross [latex]\text{I}_{W}[/latex]to the inbred to which it is more related (that is, more genetically similar) to create a breeding population, thus preserving heterosis between [latex]\text{I}_{New}[/latex] and the other parent line.
Assigning New Germplasm to a Heterotic Group
Assuming a particular heterotic pattern has been recognized and utilized, new potential breeding materials can be assigned to a relevant heterotic group through:
- Pedigree analysis
- Geographic inference
- Genetic similarity based on molecular marker profile using cluster analysis (e.g. Mumm and Dudley, 1994)
- Measurement of heterosis
- Combining ability analysis
The latter two are typically conducted using materials created through mating designs such as diallel or partial diallel, or NC Design II.
Establishing a Heterotic Pattern
Separate heterotic groups are essential for the development of hybrid cultivars. If heterotic groups have not yet been defined, there are approaches that can provide guidance on a starting point. A diallel mating design can be used to measure heterosis between pairs of lines (as demonstrated in Eberhardt, 1971). Heterotic grouping can be arbitrarily assigned to maximize heterosis between heterotic groups.
In addition, the phenotypic data can be supplemented by cluster analysis of a larger set of lines based on molecular marker profile (see Chapter 2 for guidance on cluster analysis based on molecular marker profile). The cluster analysis can be used to identify genetically similar materials, with the assumption that genetically similar lines will perform similarly in hybrid combinations. The lines that showed heterosis in hybrid combination can serve to anchor the groupings (clusters) and indicate heterotic group assignments as well as heterotic subgroup membership.
The newly established heterotic pattern can be reinforced and strengthened through the use of breeding methods such as reciprocal recurrent selection. Furthermore, one of the heterotic groups in a newly established heterotic pattern will be chosen to serve as the pool of female hybrid parents and the other as the male. Thus, selection within a heterotic group will also focus on reproductive features important to its role in hybridization. That is, lines in the female pool will likely be improved for seed set whereas lines in the male pool will be improved for traits associated with pollen shed.
Parents of Breeding Crosses Chosen Within a Heterotic Group
In developing improved hybrid cultivars, New Line Development will take place within the context of each heterotic group. That is, parents for breeding populations are chosen within, not between, heterotic groups. To maximize genetic diversity within the breeding population, parents may be chosen from different heterotic subgroups within the heterotic group. For example, the choice of parents for a breeding population to improve the female side of the pedigree in U.S. maize may involve a line from the B14 heterotic subgroup and a line from the B73 subgroup (Fig. 3).
Prospective parents within a heterotic group can be evaluated on the basis of estimated breeding value (EBV) in hybrid combination with members of the opposite heterotic group for traits involving heterosis. Whereas EBVs for pure line varieties consider the merit of lines per se, EBVs for prospective parents of improved inbreds for hybrid cultivars take complementarity into account. (For a comparison of examples, see Bernardo, 2010.)
Classes of Loci
For any given prospective parent, IW, the value of its contribution to improving a given hybrid, I1 x I2, can be assessed based on the methodology proposed by Dudley (1987).
To increase the frequency of favorable alleles in HNow, certain classes of loci are key. With inbred parents, there are eight classes of loci relative to I1, I2, and IW, as shown in Table 1.
Class of loci | I1 | I2 | IW |
---|---|---|---|
A | ++ | ++ | ++ |
B | ++ | ++ | – – |
C | ++ | – – | ++ |
D | ++ | – – | – – |
E | – – | ++ | ++ |
F | – – | ++ | – – |
G | – – | – – | – – |
H | – – | – – | – – |
Because all lines are homozygous, only one allele is present at each locus; “+” is used to indicate the dominant (favorable) allele, and “–” to indicate the recessive allele in Tables 1, 2, 3, and 5.
Which Classes of Loci are Important?
Which classes of loci are important to developing an improved hybrid?
With Classes A and H, all lines are invariant; no new favorable alleles are introduced.
If dominance is complete (a=1), then no need for improvement at Class C or D if I2 is to be improved, or at Class E or F if I1 is to be improved.
With Class G, IW has new favorable alleles not present in either I1 or I2, which is imperative.
Potential Gain / Potential Loss
If IW is crossed to I1, there is potential for loss with Class D loci, and the potential for gain with Class G loci in HNew (Table 2).
Class of Loci | I1 x IW | I2 |
A | ++ | ++ |
B | ++ | ++ |
C | ++ | — |
D | +- | — |
E | — | ++ |
F | — | ++ |
G | +- | — |
H | — | — |
If IW is crossed to I2, there is potential for loss with Class F loci and the potential for gain with Class G loci in HNew (Table 3).
Class of Loci | I1 | I2 x IW |
---|---|---|
A | ++ | ++ |
B | ++ | ++ |
C | ++ | – – |
D | ++ | – – |
E | – – | ++ |
F | – – | +– |
G | – – | +– |
H | – – | – – |
Clearly, IW needs to be a source of new favorable alleles (Class G), but the potential loss through Class D or F must be considered as well.
Dudley (1987) devised a methodology to evaluate potential new parental sources to determine which have the highest probability of producing an improved hybrid HNew when used to create breeding populations with either I1 or I2. The method also provides guidance on which parent of HNow to improve and whether to create F2 or BC1 families as a starting point.
General Principles of Choosing Parents
In general, the principles of choosing parents do not change in working with a hybrid cultivar (versus a pure line variety):
- Need sources with a high frequency of favorable alleles for the traits defined in the product target.
- Need a diversity of favorable alleles between the parents of the breeding crosses so there is genetic variability among the progeny comprising the breeding population.
In developing hybrid cultivars, there is also a need to preserve heterosis between the heterotic groups. Therefore, breeding crosses are made within heterotic groups and progeny are evaluated in terms of testcross performance to meet the need to build complementarity between heterotic groups.
Ultimately, improved hybrids illustrate strong specific combining ability.
Controlled Pollination
Of course, breeding crosses are made in such a way as to strictly control the parental contributions of pollen and ovule.
One major difference in New Line Development and New Line Evaluation between hybrid cultivars and pure line varieties is the need for controlled pollinations once the breeding populations have been formed. As we saw with soybean, once the breeding cross is made, all other pollinations are self-pollinations. With soybean, self-pollination takes place even before the complete flower opens.
With the development of hybrid cultivars, there are situations where seed produced through self-pollination is not the objective. Thus, a means to perform controlled pollinations with a specific pollen parent is needed on a fairly large scale.
Consider steps for controlled pollination in maize… see the following YouTube video by Tracy and Kaeppler at the University of Wisconsin for a demonstration:
Breeding Strategies
Let’s consider breeding strategy since this is, in large part, a function of choice of the base germplasm used and choice of parents for breeding crosses.
The pedigree breeding method is popular with hybrid cultivar development (see Chapter 2 for detail on the pedigree breeding method). Elite lines are crossed to develop a breeding population. However, in developing hybrid cultivars, progeny will be evaluated for testcross performance for any traits involving heterosis.
If the base germplasm is not particularly elite for yield performance and other non-additive key traits, recurrent selection methods may be practiced as a means to increase the frequency of favorable alleles within a heterotic group.
Recurrent Selection
Recurrent selection involves the systemic development and improvement of a breeding population. Individuals in the population are evaluated with respect to a trait(s) of interest and selected lines are intermated to form the next cycle.
In practicing recurrent selection, there are a number of mating designs that can be used to create materials for testing. Selection can take place on an individual plant basis or on the basis of family structure (i.e. half-sib families, full-sib families, S1 families), depending on the heritability of the trait(s) for which the population is to be improved.
The improved population can be used as a source of new inbreds. With any cycle, top-performing lines can be spun out for use as parents of improved hybrid cultivars and/or used as parents to develop breeding populations (e.g. implementing pedigree breeding).
Iowa Stiff Stalk Synthetic, a synthetic variety developed in 1933-34 by George F. Sprague during his tenure at Iowa State University (Sprague, 1983), is a famous case of recurrent selection. Iowa Stiff Stalk Synthetic (BSSS) was developed with 16 lines chosen for stalk strength that were intermated to form a population that was random mated for an unknown number of generations. Several important female inbreds were spun out of various cycles: B14 from Cycle 0, B37 from Cycle 0, B73 from Cycle 5, and B84 from Cycle 7. BSSS became the basis for the female side of the pedigree in U.S. commercial maize germplasm (referred to as Stiff Stalk Synthetic, SSS), and its prominence is still noted today.
Reciprocal Recurrent Selection
In the case of hybrid cultivars, reciprocal recurrent selection may be used to simultaneously improve two populations (one from each heterotic group), boosting the frequency of favorable alleles in a complementary fashion. Thus, each population is improved with respect to the other.
The following example of reciprocal recurrent selection involves three steps per cycle:
- In the first season, a plant in Population 1 (P1) is selfed (to produce S1 seed) and crossed to several random plants in Population 2 (P2). The seeds harvested from the P2 plants are bulked to form a P1 half-sib family. The self and cross procedure is also done in P2.
- In the second season, the half-sib families in P1 (crossed to P2) and P2 (crossed to P1) are evaluated in performance tests.
- In the third season, the selfed seeds from the best plants in P1 are grown and the plants are intercrossed to form the next cycle. The selfed seeds from the best plants in P2 are likewise used in recombination separately from P1.
GCA and SCA
With reciprocal recurrent selection methods, selection within each population is based on general combining ability (GCA). Variance associated with GCA measures variation among progeny with one common parent (i.e., half-sib families).
Lines representing newly improved inbreds may also be selected based on specific combining ability (SCA). Variance associated with SCA involves variation associated with female x male line interaction.
Any selection scheme based on testcross performance with the opposite heterotic group and applied to both sides of the pedigree can be considered a form of reciprocal recurrent selection. This includes pedigree breeding with selection of progeny based on testcross performance in combination with a tester line from the opposite heterotic group.
Mating Designs to Measure GCA and SCA
There are a number of mating designs which can be used to measure GCA and SCA effects, the most common of which is the diallel.
A complete diallel involves every possible paired combination of lines. Reciprocal crosses and parent lines may or may not be included in the phenotypic evaluation (Griffing, 1956). A total of [latex]\large \frac{p-1}{2}[/latex] crosses are made with p lines in a complete diallel where reciprocal crosses are bulked. To minimize the number of crosses needed for evaluation of a large number of lines, parents may be assigned to sets which are essentially mini-diallels.
In addition to providing estimates of GCA and SCA, a diallel can be used to compute estimates of heterosis. Thus, diallel can be used in establishing a new heterotic pattern; lines showing the greatest heterosis in hybrid combination are arbitrarily assigned to opposite heterotic groups.
The NC Design II is another mating design useful for obtaining estimates of GCA and SCA effects.
Vital Need for a Testing System Based on Hybrid Performance
A testing system based on hybrid performance is vital to the development of improved hybrid cultivars. After all, the improved cultivar will be exhibiting its hybrid performance to farmers and other stakeholders in the value chain.
Sufficient, accurate data on hybrid performance is essential to making a correct decision about product launch.
Choice of Tester
Before examining a testing regime suited to New Line Development and New Line Evaluation for hybrid cultivars, let’s first consider the choice of a tester to produce testcross seed for evaluation…
Since testcross evaluation is used to assess combining ability (and to estimate breeding values), the challenge is to find a tester that provides discrimination among progeny in a breeding population in keeping with the purposes of selection.
Matzinger (1953) defined a desirable tester as one that combines the greatest simplicity of use with the maximum information on the performance to be expected from tested lines when used in other hybrid combinations or grown in other environments.
Requirements of a Tester
An ideal tester maximizes differences among the genotypes being tested. That is, an ideal tester maximizes the variance among testcrosses:
[latex]\large V_{TC}=\frac{1}{2}(1+F)pq\,\big[a+d(q_{T}-p_{T})\big]^2[/latex]
The equation indicates that [latex]V_{TC}[/latex] is a function of:
- Allele frequencies in the population, p and q.
- Allele frequencies in the tester, pT and qT.
- Level of inbreeding in the population, F.
- Levels of dominance, d.
- The value, a, which is equal to half the difference between genotypic values of the two homozygotes (i.e. additive effect) across loci affecting trait performance.
Note that for any level of dominance, the quantity
[latex]\big[a+d(q_{T}-p_{T})\big]^2[/latex]
is maximized when qT = 1. That is, the tester is fixed for the recessive allele at most underlying loci.
The recessive allele from the tester does not mask the effect of a favorable, dominant allele contributed by the progeny. However, it also suggests poor performance per se of the tester line.
Also note that if d =0, then the tester has no effect. However, most loci involved with the expression of heterosis are expected to have some level of dominant gene action.
Comparison of Tester Types
Hallauer and Lopez-Perez (1979) compared five types of testers in maize for their value in assessing lines from Iowa Stiff Stalk Synthetic (BSSS) at early (S1) and late (S8) stages of inbreeding. The testers were compared as to their testcross variance, [latex]V_{TC}[/latex], and mean performance, µT, for grain yield, a highly heterotic trait (Table 4).
Testers evaluated in combination with BSSS included:
- The BSSS population itself.
- BC13(S)C1, an improved version of the BSSS population.
- BSSS-222, a low-yielding inbred selfed out of BSSS.
- B73, a high-yielding inbred selfed out of BSSS Cycle 5.
- Mo17, a high-yielding inbred line from the opposite heterotic group.
Tester | Description | S1 [latex]V_{TC}[/latex]
(t/ha) |
S8 [latex]V_{TC}[/latex]
(t/ha) |
S1 μT
(t/ha) |
S8 μT
(t/ha) |
---|---|---|---|---|---|
BSSS | Population itself | 0.18 | 0.42 | 5.79 | 5.69 |
BC13(S)C1 | Improved BSSS population | 0.11 | 0.34 | 6.95 | 6.81 |
BSSS-222 | Poor BSSS Inbred | 0.22 | 0.39 | 6.03 | 5.89 |
B73 | Elite BSSS inbred | 0.04 | 0.26 | 7.29 | 7.21 |
Mo17 | Elite non-BSSS inbred | 0.26 | 0.30 | 7.81 | 7.78 |
Conclusions:
- More differentiation among progeny was possible with S8 vs S1 testcrosses (i.e., greater F in the progeny highlighted the allele frequencies) despite consistency in performance levels.
- The poor performing testers generally led to higher [latex]V_{TC}[/latex] (e.g., BSSS-222 vs B73; BSSS vs BC13(S)C1). Note that all of these testers are related to BSSS.
- In early testing, [latex]V_{TC}[/latex] was maximized by the Mo17 tester, the elite line from the opposite heterotic group.
Clearly, the Mo17 tester is highlighting differences among BSSS lines at loci for which Mo17 is deplete of favorable alleles! Also, the Mo17 tester provided the best mean performance for yield in both early and late testing. This is a tremendous advantage because a poor tester cannot be considered as a potential parent of an improved hybrid; an elite line from the opposite heterotic group can.
Poor vs. Elite Tester
Thus, there are two types of testers that can maximize [latex]V_{TC}[/latex]:
- A poor-performing tester, presumably due to a low frequency of favorable alleles
- An elite inbred tester from the opposite heterotic group, especially lines that could potentially serve as parents of an improved hybrid.
With the second option, yield trials to evaluate testcross performance are identifying the best lines for one side of the pedigree, and the yield trials also capitalize on SCA to serve in identifying top-performing hybrid combinations as potential new cultivars!
At the Genotypic Level
Let’s dive deeper…
Consider the following classes of loci in S8 progeny resulting from a breeding cross (Dudley, 1984) (Table 5).
Class of Loci | Breeding
Inbred 1 |
Cross
Inbred 2 |
Poor Tester | Elite Tester |
i | ++ | ++ | — | ++ or — |
j | ++ | — | — | — |
k | — | ++ | — | — |
l | — | — | — | ++ |
The poor tester has recessive alleles at most or all classes of loci, whereas the elite tester from the opposite heterotic group is homozygous for the dominant favorable allele at Class ‘l ‘ loci for which the progeny are depleted of favorable alleles. The elite tester may be homozygous for the dominant favorable allele at Class ‘i ‘ loci, but these alleles don’t contribute to better performance since the progeny are already fixed for the favorable allele.
Progeny are segregating for Classes ‘j ‘ and ‘k’ only. Therefore, these are the loci affecting [latex]V_{T}[/latex] . Since neither tester is contributing to these loci, [latex]V_{TC}[/latex] is the same with both testers.
However, the elite tester from the complementary heterotic group contributes at Class ‘[latex]l[/latex]‘ loci, increasing µT.
Take-Home Message
Bottom line: an elite inbred(s) from the complementary heterotic group is an ideal tester.
All progeny are compared on the same basis using a common tester or a common set of multiple testers. It will highlight real genetic differences among progeny under evaluation and, thus, serve to optimize the response to selection!
In addition, potential new single-cross hybrid cultivars may be detected in the evaluation process.
A Word About Producing Testcross Seed
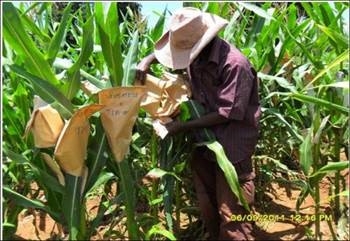
To produce testcross materials for trials, progeny can be crossed to a tester from the opposite heterotic group through the use of a topcross mating design. Generally, a topcross design is carried out in an isolated field; families of the breeding population are grown in separate rows with the male flower removed before pollen shed. The tester, grown in rows placed intermittently through the field, serves as the pollen parent in the testcross, with pollen transmitted to progeny rows through wind, insects, or other means. The field isolation prevents stray pollen from contaminating the testcross seed.
Another alternative is to produce testcross seed through paired-row crosses. Each progeny (or progeny family) is represented in one row, with the tester grown in the adjacent row. Silks are protected to prevent inadvertent pollen ahead of hand-pollination and pollen is collected and distributed manually (Fig. 7); thus, field isolation is not required. Seed produced on plants in either of the paired rows can be bulked to represent the testcross seed associated with that progeny.
Product Target Example: Maize Single-cross Hybrid
Consider the following product target for a breeding program focused on single-cross maize:
The product target describes the “what” and the “for where.”
Where is the improved hybrid intended for? Describe the target market region specified in this product target.
The target market region also implies what population of environments?
Click here to reveal the answer
Environments representing geographic location and also the season, maturity zone, altitude, soil types and farmer practices.
What characteristics does this product target imply?
- White grain.
- High grain yield.
- Tolerance to moisture stress levels common to non-irrigated production.
- Good standability (minimal/no stalk lodging or root lodging).
- A strong “disease package” for this region might include resistance to.
- Grey leaf spot (Cercospora zeae-maydis and Cercospora zeina)
- Common rust (Puccinia sorghi)
- Diplodia ear rot (Stenocarpella maydis)
- Medium maturity.
- Bt event (e.g., transgenic event which imparts resistance to stalk borers).
- RR event (e.g., transgenic event which imparts tolerance to glyphosate herbicide such as Roundup®).
Possible Secondary Traits to Collect
What characteristic(s) would be a good indicator of tolerance to moisture stress in maize?
Yield evaluations will be conducted in the target market region, which will provide an indication of response to any level of drought encountered in that environment.
In addition, another indicator could be considered: anthesis-silking interval (ASI). ASI is the number of days between the date when 50% of plants are shedding pollen and the date when 50% of plants are showing emerged silks. ASI is an external indicator of the metabolics underlying stress tolerance. Large ASI in maize is a sign of reduced partitioning to the ear, resulting in slow spikelet growth which affects kernel number. ASI is highly correlated with barrenness which, like kernel number, is a contributing factor to grain yield. ASI is a clear indicator of stress at flowering, which is the most vulnerable time for yield loss due to drought under “occasional drought.” ASI not only signals moisture stress, it is a good indicator of other abiotic stresses such as nitrogen deficiency.
Is the use of ASI to indicate tolerance to moisture stress an example of a secondary trait?
Click here to reveal the answer
Yes!
Measuring Performance of Desired Characteristics
The specific levels of the desired characteristics, the selection thresholds, and the way the characteristics will be measured can be detailed after the target characteristics. Any protocols to be used in trait evaluation can be specified.
For example (Table 6):
Characteristic | Measurement Standard | Threshold Level / Range |
---|---|---|
White grain | Visual; 1 = white | 1 |
High grain yield | Machine harvest; grain weight on a 12.5% moisture basis, expresses per unit of land | 10% greater than Hybrid X |
Tolerance to moisture stress | ASI (anthesis-silking interval) calculated as the difference between date of 50% silk emergence. Compute the number of days from planting to anthesis and number of days from planting to silking, from record of anthesis date and silking date. | -2 ≤ x ≤ 2 |
Stalk lodging | Estimated % affected plants per plot | ≤ 5% |
Root lodging | Estimated % affected plants per plot | ≤ 5% |
Gray leaf spot | 1-9 disease scoring scale, 1=no infection, 2=very low infection, 3=low infection. Protocol for pathogen inoculation and disease screening. | Score ≤ 2 |
Common rust | 1-9 disease scoring scale, 1=no infection, 2=very low infection, 3=low infection. Protocol for pathogen inoculation and disease screening. | Score ≤ 3 |
Diplodia ear rot (Stenocarpella maydis) | 1-9 disease scoring scale, 1=no infection, 2=very low infection, 3=low infection. Protocol for pathogen inoculation and disease screening. | Score ≤ 2 |
Medium maturity | Growing degree days from planting to physiological maturity | 110-115 day range (warm areas) |
Bt event (YieldGard II) | MON89034 expression | Pre-determined level of Lepidopteran resistance |
RR2 event (Roundup Ready 2) | MON88017 expression | Pre-determined level of glyphosate tolerance |
Note that Bt and RR2 events require licensing by the breeder’s organization. The events listed here are examples.
Example Commercial Maize Hybrid Improvement Program
Consider a suitable testing regime to identify hybrids that meet the example product target, starting with a commercial maize hybrid breeding program as an example. Note that this program serves a market region with one growing season (Summer) (Table 7):
Season1 | Activity |
Summer 0 | Make breeding crosses |
Winter 0 | Self or BC |
Summer 1 | 1. Grow 40 F2 or BC1 populations (i.e. S0 generation) with 200+ plants each
2. Select S0 plants in each population based on plant type, disease and insect resistance, marker genotype, etc. 3. Self and testcross 100 selected S0 plants in each population to an inbred tester |
Summer 2 | 1. Discard ≤5 F2 or BC1 populations based on performance data on the parents, obtained from the previous year
2. Grow 3500 S0 testcrosses in unreplicated trials at 2-4 locations 3. In each S1 family, self ≥3 plants to obtain two S2 subfamilies per S1 family. Discard S1 families that appear inferior. 4. Select the best 350 families based on their S0 testcross performance. |
Winter 2 | 1. Cross 700 S2 families to each of two inbred testers
2. Self S2 families to obtain S3 families |
Summer 3 | 1. Evaluate 1400 S2 testcrosses in unreplicated trials at 6-10 locations
2. Select 8-15 S3 families based on their S2 testcross performance; code lines |
Winter 3 | Self the selected S3 families to obtain S4 seeds of new inbreds |
Summer 4 | 1. Cross each new inbred with 6-10 elite inbreds
2. Self the S4 lines to obtain S5 seeds of new inbreds |
Summer 5 | 1. Yield trials of experimental hybrids at 15-40 locations
2. Self the S5 lines to obtain S6 seeds of new inbreds |
Summer 6 | 1. Yield trials of advanced hybrids at 20-75 locations
2. On-farm strip tests (i.e. 150-300 m2 plots) at 30-500 locations |
Summer 7 | On-farm strip tests of pre-commercial hybrids at 50-1500 locations |
Fall | Release 0-2 new hybrids |
1 Number after season indicates the year in the development pipeline. Note: This program serves a market region with one growing season per year (Summer). Winter indicates off-season activities.
Salient Features
We observe the following with respect to the Example Commercial Maize Hybrid Improvement Program:
- The main focus of testing is grain yield in this example; high grain yield is obviously the trait of the utmost priority in producing new hybrids (in this case).
- Selection for highly heritable traits is performed in early generations (selection among individual S0 plants is indicated in this example).
- A large number of breeding populations are created, with about 50-100 plants per population. This would include breeding crosses made with bot heterotic groups (e.g., 40 female populations and 40 male populations).
- All yield trials are conducted using testcross materials.
- Yield trials are initiated with a large number of S0 testcross lines, each representing a different S0 family.
- Selection intensities are high (only a small proportion of the tested families are advanced to the next generation of testing).
- The number of elite inbred testers crossed to each family under evaluation for yield increases as the number of tested families decreases.
- The number of locations for yield testing increases as the number of tested families decreases.
- Lines that are selected after Summer 3 yield trials (second year of yield testing) are coded as “experimental” and progressed as S4’s to wide-area testing the following growing season; once coded lines are coupled with elite inbred testers, it is the hybrid that is under selection, not the S4 line.
- Wide-area testing includes “on-farm trials” managed by potential seed customers in the second year.
- Overall, there are at least four years of comprehensive performance testing: four seasons of “research” yield trials, two of which are wide-area testing.
- A pre-commercial stage to acquaint farmers with potential new products is featured in the fifth year of testing; this year could also include National Performance Trials required for registration of a new variety.
- Because maize plants can be self-pollinated and the tassel is used for cross-pollination at the same time, nurseries require a setup that facilitates producing both types of seed.
Adaptation Needed to Meet the Stated Product Target
Given this example of New Line Development and New Line Evaluation from commercial maize, what modifications in the breeding and testing regime are needed to fit the evaluation and selection required to achieve the example product target?
Note that after seven years of hard work and significant resource expenditure, it is possible to wind up with zero new, improved hybrids! How will your pipeline design of New Line Development and New Line Evaluation minimize the potential for failure?
Male Sterility Required for Hybrid Seed Production
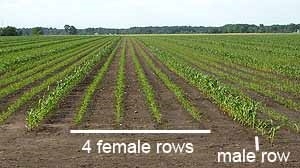
An important consideration in the development of hybrid cultivars is the need to affect male sterility during hybrid seed production in a cost-effective manner. Controlled pollination on a large scale is essential to ensure that the hybrid seed being produced for the farmer is a product of the intended cross between the elite female inbred (from the female heterotic group) and elite male inbred (from the male heterotic group). In particular, prevention of self-pollination by the female inbred plants maximizes the probability that the pollen parent is the desired male inbred in a wind-pollinated crop such as corn.
Hybrid production fields are arranged to include a predetermined ratio of female inbred (i.e. seed parent) rows to male inbred (i.e., pollen parent) rows (Fig. 8). Common ratios are 4:1, 4:2, 6:2, depending on the pollen dissemination range. Hybrid production fields are grown in isolation to avoid the intrusion of foreign pollen.
Ways to Control Pollination
Control of pollination can be facilitated physically through:
- Separate female/male plants (i.e., the crop species is dioecious)
- Manual or mechanical means of removing male flowers or male reproductive structures. In corn hybrid seed production, physical removal of the male flower of the maize plant, referred to as “detasseling”, requires a large short-term labor force (Fig. 9).
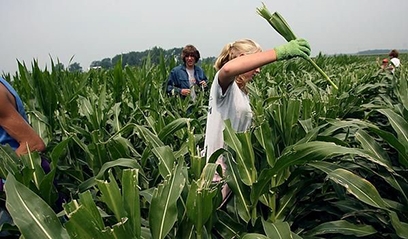
Alternatively, male sterility (MS) systems in the plant can be utilized to prevent pollen shed in female inbred parent, which can lead to selfed rather than hybrid seed.
There are several types:
- Genic MS.
- Environmentally-induced MS.
- Cytoplasmic MS.
- Transgenic MS.
- Chemically-induced MS.
Genic Male Sterility
Genic MS is controlled by nuclear genes. It is often determined by a recessive allele at a single locus.
Genic MS was first reported by L.A. Eyster (1921) in maize. Today nearly all identified nuclear MS genes in maize have been mapped. Furthermore, MS can be affected by mutation.
Examples of genic MS include:
- The ms45 allele in maize: this recessive allele in a homozygous state results in MS, whereas fertility is restored by a single copy of the dominant allele, Ms45.
- The suppressor-mutator (Spm) transposable element system (Brar et al. 1987).
Environmentally-induced Male Sterility
Some nuclear MS genes can be induced under certain environmental conditions. Thermo-sensitive and photoperiod-sensitive nuclear genes have been used in rice, for example, to facilitate hybrid production. This enables the MS female inbred lines to be maintained through self-pollination in environments not conducive to MS induction, while hybrid production is carried out under conditions that promote MS.
This type of two-line system (male-sterile female line, restorer line) is not without issues as weather conditions can be unpredictable and inconsistent. Temperature fluctuations may cause “leaks,” where some viable pollen is produced, or even complete reversal to a fertile state.
Cytoplasmic Male Sterility
Compared to the normal maize inbred, the CMS-version of inbreds does not exert anthers and therefore does not shed pollen (Fig. 10), a trait that is exploited in hybrid seed production.
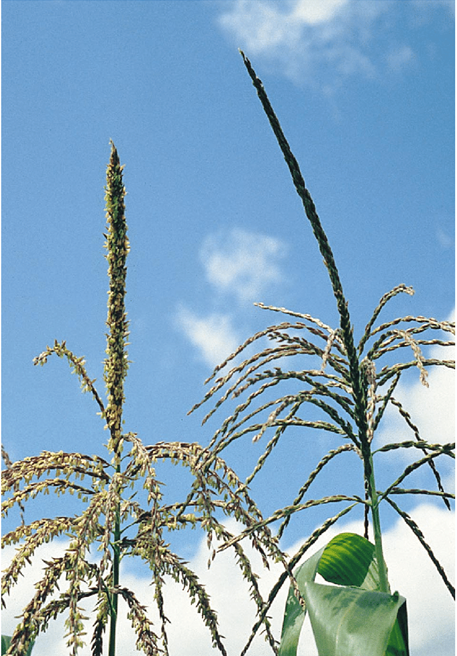
Cytoplasmic male sterility (CMS) has been observed in more than 150 species. It is transmitted exclusively by the female parent as a function of the mitochondrial genome. Restoration of fertility relies on nuclear genes that suppress cytoplasmic dysfunction. Each MS cytoplasm has its own “key” to restore fertility in the form of nuclear gene(s) referred to as Rf (Restorers of fertility) (Fig. 11).
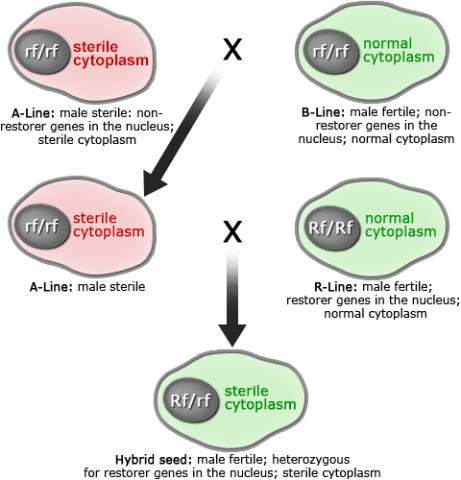
Some examples of CMS systems in maize include:
- CMS T-type: Dominant Rf1 and Rf2 genes, both required.
- CMS S-type: Dominant Rf3 gene.
- CMS C-type: Dominant Rf4 involved, maybe others.
CMS has been observed in more than 150 plant species and similar CMS systems have been detected in many crops.
Maintaining the CMS Inbred Line
With CMS systems, the need to maintain the MS inbred is accomplished through the use of a maintainer line, which is genetically identical to the MS inbred except that it does not have the MS cytoplasm. Both the MS inbred and its maintainer line harbor the recessive MS restorer allele (denoted r). When the MS female inbred is crossed to the male inbred containing the nuclear fertility restorer gene (denoted R ) in hybrid production, the resulting F1 seed for farmers is male fertile despite having the MS cytoplasm.
Note that this system relies on certain genetic elements on both sides of the hybrid pedigree. Such a system is referred to as a three-line system (CMS line, maintainer line, restorer line) (Fig. 12).
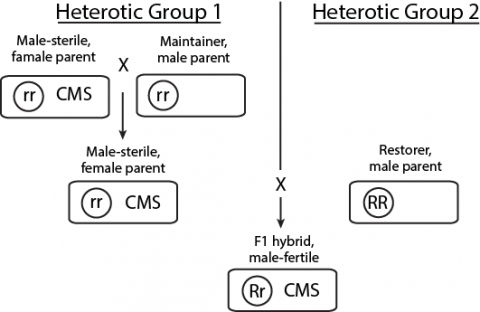
Choosing a CMS System
When considering the use of a CMS system, it is critical to compare options and explore the possibility of the negative impact of aberrant mitochondrial genes on hybrid performance.
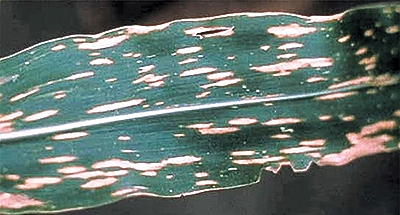
Photo courtesy of David B. Langston, University of Georgia.
The U.S. corn crop was nearly wiped out in 1970 due to the widespread use of T-CMS, which is susceptible to Southern corn leaf blight (Fig. 13) caused by pathogen Bipolaris maydis (also known as Cochliobolus heterostrophus).
Transgenic Male Sterility
Some systems of male sterility have been created through genetic engineering. For example, DuPont Pioneer (now Corteva Agrisciences) has employed an innovative proprietary Seed Production Technology (SPT) system involving transgenic event DP-32138-1 in maize (Commuri et al, 2009), which relies on the zm-aa1 gene to render pollen inviable (Fig. 14). The event also includes an Ms45 gene, which restores fertility in an ms45/ms45 background as well as a red fluorescent protein (DsRed2 ) gene, which serves as a marker for transgenic seeds. The transgenic male-fertile maintainer line is used to produce non-transgenic seed of the female parent of the hybrid (transgenic seed is also produced due to segregation for the event and this seed is used to propagate the maintainer line). The non-transgenic MS female plants are used in hybrid production to create non-transgenic hybrid seed. Fertility is restored in the F1 seed through its Ms45/Ms45 male parent. Thus, the transgenic element of the system does not enter the value chain or the food system.
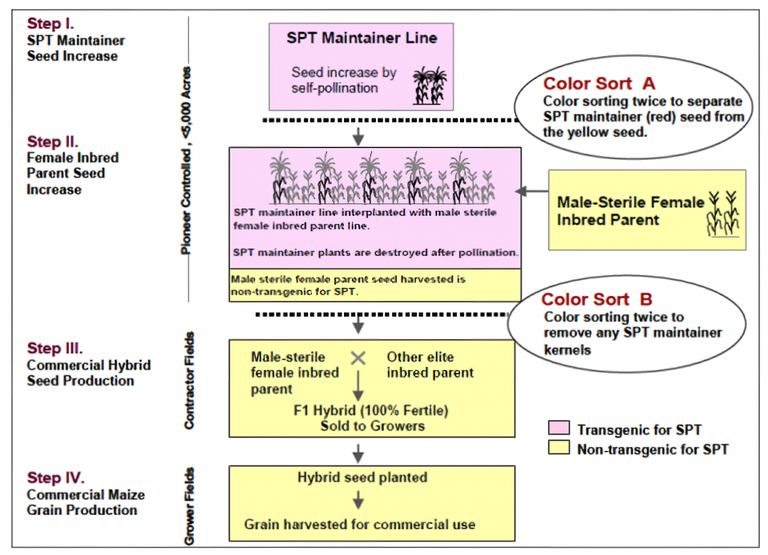
A transgenic MS system in rice utilizes a mutant allele of nuclear gene OsNP1 (Oryza sativa No Pollen 1), which encodes a regulator gene controlling tapetum degeneration and pollen exine formation. The osnp1 mutant exhibits male sterility, which is insensitive to environmental conditions (Chang et al., 2016).
Chemically induced Male Sterility
MS has been induced in a number of crops including rice, sugarbeet, wheat, cotton, rapeseed, canola, cucurbits, tomato, and onion using chemical hybridization agents (CHAs) such as male gametocides (e.g., gibberellic acid, maleic hydrazide, naphthalene acetic acid, ethereal). Many CHAs, including classes of sulfonylureas and imidazolinones, inhibit acetohydroxyacid synthase (AHAS), an important enzyme in amino acid biosynthesis. A MS system in rapeseed utilizes a sulfonylurea-resistant mutant to maintain normal male fertility in the pollen parent during hybrid production despite herbicide application to the entire field (Li et al., 2015).
Chemically induced MS can be coupled with transgenic MS systems as a means to “switch on” expression at a desired time. Monsanto’s proprietary MS system in maize based on event MON 87427 (Breeze et al, 2010) utilizes glyphosate herbicide (e.g., Roundup®) as a means to induce MS just prior to tassel development. The seed parent in hybrid production has been converted to MON 87427 which protects all plant tissues except tapetum cells and pollen grains against the effect of glyphosate. Thus, herbicide application affects only these tissues. Note that the pollen parent in hybrid production must be protected against damage from glyphosate application through incorporation of a glyphosate-tolerant event which is expressed in all plant tissues.
The key with use of chemically induced MS is that female fertility is not affected.
Incorporating MS System Elements Through Breeding
There are many options available to facilitate hybrid production through controlled pollination. MS systems are key to producing genuine F1 seed of the improved inbreds the breeder has worked diligently to develop.
Although hybrid production to produce F1 seed for farmers takes place downstream of New Line Development, New Line Evaluation, and Trait Integration, the elements of the MS system used must often be integrated through breeding efforts. Thus, choice of a MS system is a decision affecting the design of the product pipeline. Introgression of MS system elements will be discussed in more depth in Chapter 5 on Trait Introgression.
Single-cross Hybrids
This chapter has focused on the design of New Line Development and New Line Evaluation for improvement of hybrid cultivars. The focus has been on single-cross hybrids whereby F1 seed is produced by crossing two genetically unrelated inbred lines, one from the female heterotic group and one from the male heterotic group.
Each seed has a genetic complement from each parent. At every locus where the two inbred parents possess different alleles, the single cross hybrid is heterozygous. All F1 seeds are genetically identical and plants are uniform.
Double-cross Hybrids and Three-way Hybrids
When the heterotic pattern is young and inbreeding depression in hybrid parents is still an issue, the use of double-cross hybrids can offer an advantage. Double-cross hybrids involve four distinct inbreds as parent lines and require two steps to create hybrid seed for farmers. Two inbreds from the female heterotic group (P1 and P2) are crossed and two inbreds from the male heterotic group (P3 and P4). Then, the F1 seed produced by the female parents is crossed to the F1 seed produced by the male parents. The vigor of plants serving as parents of hybrid seed production is improved due to within-heterotic-group variability (i.e. the parental lines perform better), boosting hybrid seed yield. The hybrid seed for farmers exhibits between-group heterosis.
A three-way cross is a variation on double-crossing: the hybrid seed for farmers involves a single-cross as one parent and an inbred line as the other parent. Typically, the single-cross parent is the female parent of the hybrid.
Disadvantages of Multi-cross Hybrids
Although use of double-crosses and three-way crosses can overcome effects of inbreeding depression to improve seed yields in hybrid production, there are disadvantages to be weighed as well. An extra step is required in hybrid production which demands additional time and resources in product development. Furthermore, the hybrid seed for farmers is not genetically uniform. Thus, the improved multi-cross hybrid is not expected to yield as well as a single-cross hybrid since SCA is not maximized.
Bottom line: Any new prospective cultivar must be able to be reproduced efficiently and cost-effectively!
References
Bernardo, R. 2010. Breeding for Quantitative Traits in Plants, 2nd ed. Stemma Press, Woodbury, MN.
Brar, G.S., O.N. Middleton. 1987. Genic male-sterile maize. United States Patent. Patent number: 4,654,465.
Breeze, M.L., K.S. Crowley, A. Deffenbaugh, J.R. Groat, G.D. Grothaus, D.I. Gustafson, T.O. Koyejo, T.C. Lee, D. P. Phillion, D. A. SaltmirGs, N.K. Scanlon, M.R. Starke, J. Soteres, J.E. Whitsel, M.A. Widemann. 2010. Petition for the Determination of Non-regulated Status for MON 87427 Maize with Tissue-Selective Glyphosate Tolerance Facilitating the Production of Hybrid Maize Seed. http://www.aphis.usda.gov/brs/aphisdocs/10_28101p.pdf
Chang, Z., Z. Chen, N. Wang, G. Xie, J. Lu, W. Yan, J. Zhou, X. Tang, X.W. Deng. 2016. Construction of a male sterility system for hybrid rice breeding and seed production using a nuclear male sterility gene. PNAS 113(49): 14145-14150. doi: 10.1073/pnas.1613792113
Commuri, P., N. Weber, T. Rood, R. Townsend. 2009. Petition for the Determination of Nonregulated Status for Maize 32138 SPT Maintainer Used in the Pioneer Seed Production Technology (SPT) Process. http://www.aphis.usda.gov/brs/ aphisdocs/08_33801p.pdf
Dudley, J.W. 1987. Modification of methods for identifying inbred lines useful for improving parents of elite single crosses. Crop Sci. 27: 944-947.
Dudley, J.W. 1984. A method for identifying populations containing favorable alleles not present in elite germplasm. Crop Sci. 24: 1053-1054.
Eberhardt, S.A. 1971. Regional maize diallels with US and semi-exotic varieties. Crop Sci. 11: 911-914.
Eyster, L.A. 1921. Heritable Characters of maize: VII Male sterile. J. Heredity 12: 138-141.
Griffing, B. 1956. Concept of general and specific combining ability in relation to diallel crossing systems. Australian J. Biol. Sci. 9: 463-493.
Hallauer, A.R., E. Lopez-Perez. 1979. Comparisons among testers for evaluating lines of corn. Proc. Corn Sorghum Ind. Res. Conf. 34: 57-75.
Matzinger, D.F. 1953. Comparison of three types of testers for the evaluation of inbred lines of corn. Agron. J. 45(10): 493-495.
Mumm, R.H., J.W. Dudley. 1994. A classification of 148 U.S. maize inbreds: I. Cluster analysis based on RFLPs. Crop Sci. 34:842-851.
Shull, G.H. 1952. Beginning of the heterosis concept. p14-48. In Heterosis. J.W. Gowen (ed). Iowa State College Press, Ames, IA.
Shull, G.H. 1909. A pure-line method in corn breeding. Rep. Am. Breeders Assoc. 5: 51-59.
Shull, G.H. 1908. The composition of a field of maize. Rep. Am. Breeders Assoc. 4: 296-301.
Sprague, G.W. 1983. Heterosis in maize: Theory and Practice, p 47-70. In R. Frankel (ed). Heterosis: Reappraisal of theory and practice. Springer-Verlag, New York, NY.
How to cite this chapter: Mumm, R.H. (2023). New Line Development and New Line Evaluation Single-cross Hybrid. In W. P. Suza, & K. R. Lamkey (Eds.), Cultivar Development. Iowa State University Digital Press.
Increased vigor of progeny resulting from mating of genetically dissimilar parents, or more simply, the superiority of a hybrid over either of its parents.
An average performance of an individual in a series of crosses with members of the opposite heterotic group.
The performance of a line in a specific hybrid combination.
A cross done to determine the genotype of the parent or to identify linkage between genes. Testcross involves a hybrid combination with a "tester" line of the opposite heterotic group.
Carrier of male gamete.
Plant ovary containing the female germ cell which, after fertilization, becomes the seed.
A complete flower contains both the male and female reproductive structures (stamen and pistil).
The condition of maize plants with stalks broken below the ear.
The condition of plants with stalks inclining by more than 45 degrees.
The number of days from sowing to grain ripening, which is indicated by a black internal abscission layer at the base of the kernel in maize.
The failure of the corn plant to produce an ear.
A term used in the seed industry to indicate the recognized elite status of a breeding population progeny. Typically, coded lines are entered into a wider scope of testing, which may be conducted in two stages of progression. In the first stage, coded lines are called "experimental" varieties; passing this stage, lines are called "advanced" varieties. Even if coded lines don’t make it to commercial release, these may be considered as prospective parents in creating new breeding populations.
(1) Plant species having male and female reproductive organs in separate individuals.
Staminate and pistillate flowers are on different plants of the same species.
(2) Species with separate female and male flowering plants
A type of male sterility (MS) system of hybrid production involving a male-sterile line and a restorer line, which results in male-fertile F1 seed.