Remote Muscular Control: The Human-Human Interface
Karri Haen Whitmer
In the previous exercises we have explored how we can record data from muscular contraction with an electromyogram (EMG) and how we can initiate muscle contraction by using stimulating electrodes. We first looked the EMG in the reflexes lab, where we measured the involuntary contraction of muscle in response a tendon tap. Here, we were able to determine the relative strength of the muscular response by measuring the EMG amplitude of the muscle contraction wave. In this activity, the muscular response determined by an integrating center in the spinal cord. Next, nerve stimulation and EMG concepts came together in the Nerve Conduction Velocity (NCV) Test where we stimulated the ulnar nerve with stimulating electrodes and recorded the timing of the downstream muscle contraction using EMG to determine nerve conduction velocity. We looked at the EMG again when we measured the intensity of voluntary muscle contraction in the no-contact arm wrestling activity. In this case, the EMG showed complex patterns as the muscle response was determined by higher brain centers.
Both of these techniques are powerful and each has a broad array of applications in the clinical setting. Using these techniques, could we create a method for remote muscular control?
The pathway for normal voluntary muscle control starts with motor neurons firing in the brain called upper motor neurons. These neurons synapse with a motor neuron in the spinal cord that makes up part of the spinal nerve; this neuron is called a lower motor neuron. An important point is that in order to get any signal from the brain to the body’s skeletal muscles, the pathway of the motor neurons must descend through the spinal cord to get to the muscles. The major descending pathway that connects upper motor neurons from the brain to the lower motor neurons that emerge from the spinal cord, which synapse with skeletal muscle is called the corticospinal tract (Fig 1).
The corticospinal tract is composed of two neurons, the upper motor neuron and the lower motor neuron. The upper motor neuron has its cell body in the primary motor cortex of the frontal lobe and synapses on the lower motor neuron, which is in the ventral horn of the spinal cord and projects to the skeletal muscle in the periphery.
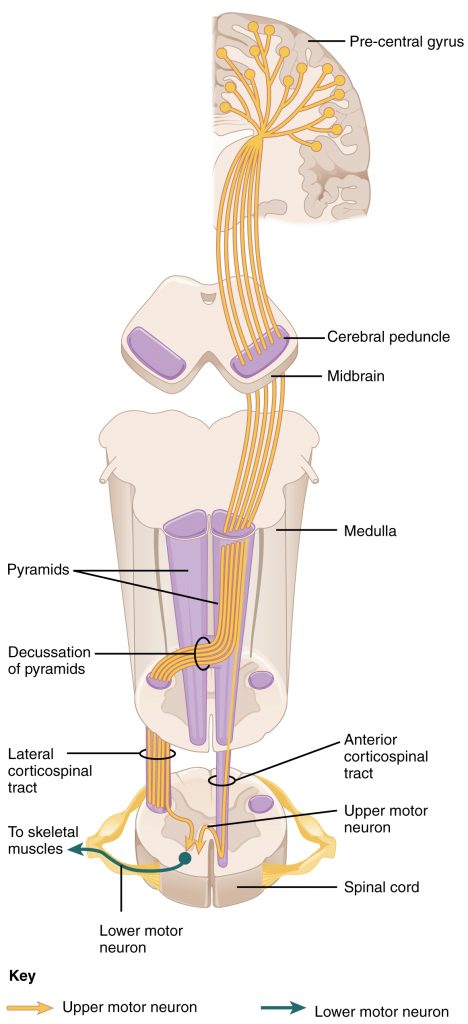
When the spinal cord is transected (cut across), such as the case of a patient in a severe car accident, the pathway is no longer intact and the upper motor neurons can no longer synapse with the lower motor neurons. Spinal cord transection injuries result in paralysis. The extend of the paralysis depends on the location of the spinal lesion. Lesions higher on the spinal column will result in more extensive loss of muscular control than lesions lower on the spinal column (Fig. 2).
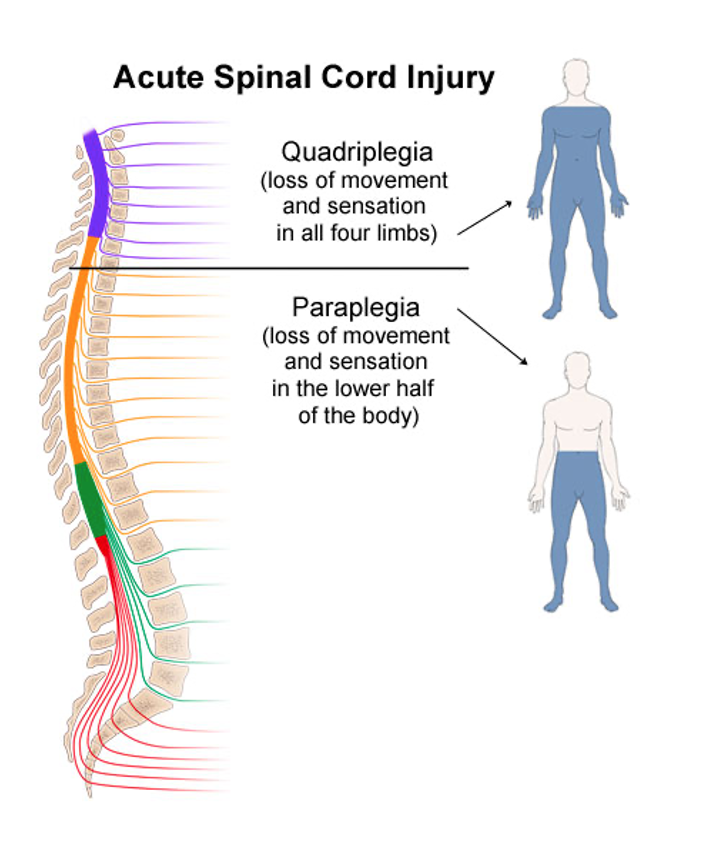
Patients with quadriplegia or other motor neuron diseases may rely strongly on assistive technologies to regain function. These commonly include tongue-controlled wheelchairs and an array of eye tracking and voice-recognition technologies that help patients control computers and smartphones. Even wearable machines like powered exoskeletons can assist users in regaining leg and arm control.
Modern prosthetic devices may be controlled by the patient’s own neural activity (indirectly via an EMG) or the device may move in response to AI driven technology. Some new devices even respond to a patient’s brainwaves through electrodes on or beneath the scalp.
In the laboratory we can simulate a major spinal injury by using the EMG and nerve stimulating electrodes by recording Subject 1’s muscle movement with the EMG, then processing this information into a signal that fires the nerve stimulators on Subject 2 (Human-Human interface; Fig. 3). In this way, we have proof of concept that a brain does not need to be linked though the spinal cord to musculature in order to activate a remote muscle group or prosthetic device. This kind of study is also proof of concept that the body (human or other animal) can be remotely “hacked,” given the proper equipment. The result of the Human-Human interface: the voluntary arm movement of Subject 1 controls the involuntary arm movement of Subject 2.
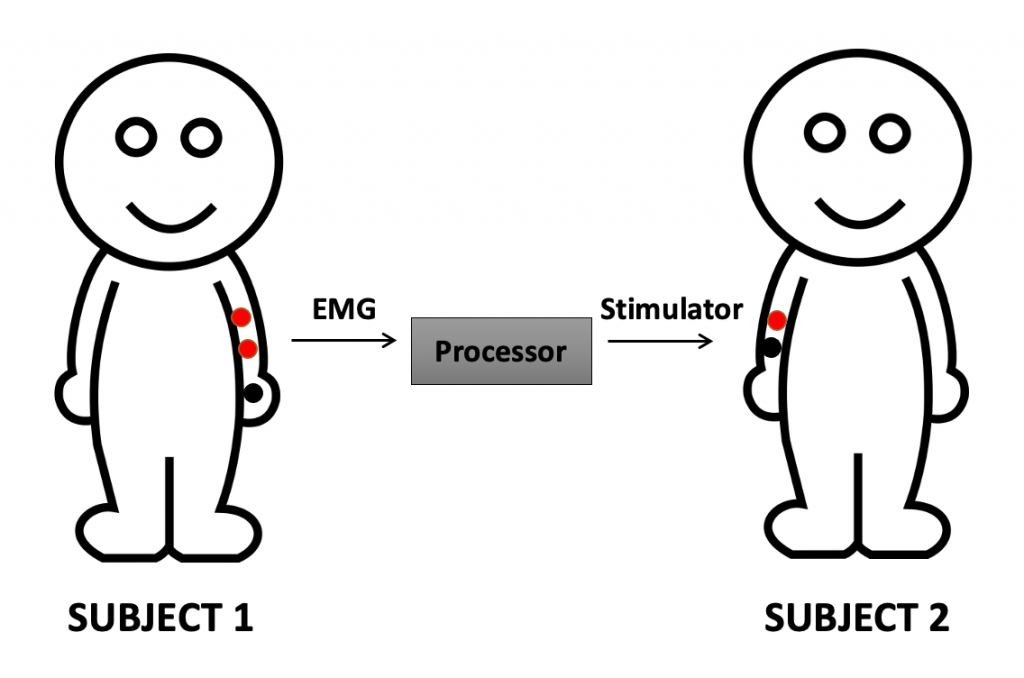
Key Points
- The human-human interface uses EMG and a nerve stimulator to allow one subject to control another subject’s arm movement.
- The methods for this activity are found in the Canvas modules.
Synthesizing concepts: muscle stimulation and contraction
Excitation and contraction coupling[1]
The sequence of events that result in the contraction of an individual muscle fiber is the same whether the stimulus is internal (from the in vivo activation of a motor neuron) or artificial (transcutaneous stimulation of a motor neuron along its axon). The process begins at the neuromuscular junction (NMJ) with the release of neurotransmitter, ACh, from the motor neuron innervating that fiber. The local membrane of the fiber will depolarize as positively charged sodium ions (Na+) enter, triggering an action potential that spreads to the rest of the membrane which will depolarize, including the T-tubules. This triggers the release of calcium ions (Ca++) from storage in the sarcoplasmic reticulum (SR). The Ca++ then initiates contraction, which is sustained by ATP (Fig. 4). As long as Ca++ ions remain in the sarcoplasm, and as long as ATP is available to drive the cross-bridge cycling and the pulling of actin strands by myosin, the muscle fiber will continue to shorten to an anatomical limit.
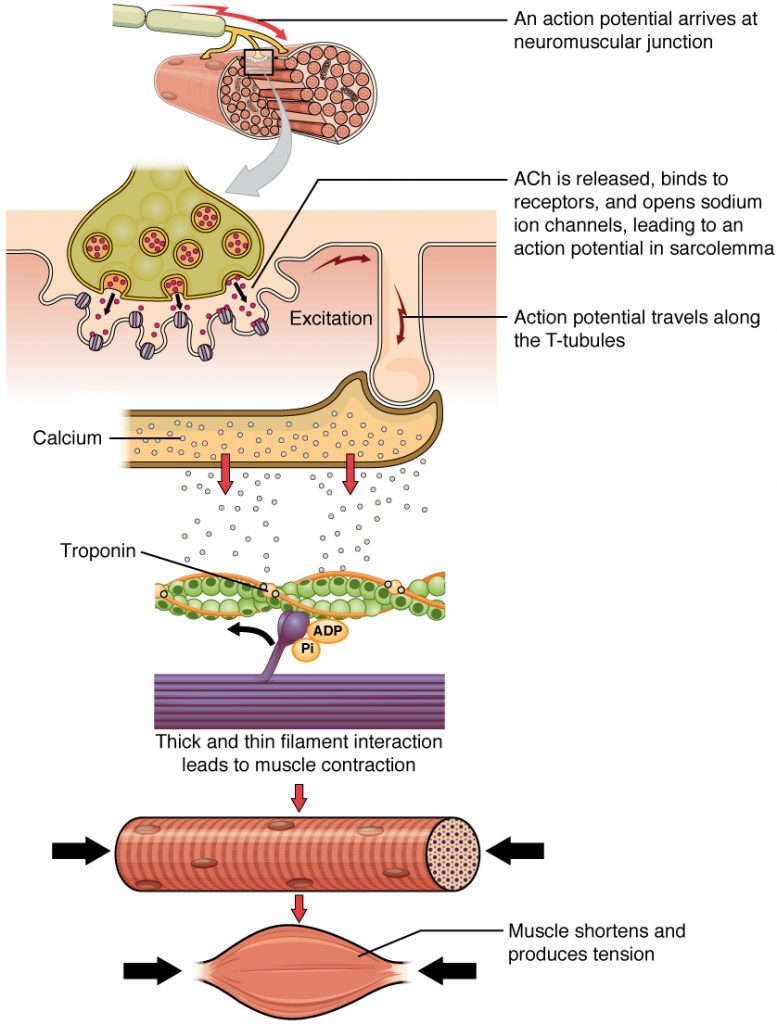
The contraction of a striated muscle fiber occurs as the sarcomeres, linearly arranged within myofibrils, shorten as myosin heads pull on the actin filaments. When signaled by a motor neuron, a skeletal muscle fiber contracts as the thin filaments are pulled and then slide past the thick filaments within the fiber’s sarcomeres. This process is known as the sliding filament model of muscle contraction. The sliding can only occur when myosin-binding sites on the actin filaments are exposed by a series of steps that begins with Ca++ entry into the sarcoplasm.
Tropomyosin is a protein that winds around the chains of the actin filament and covers the myosin-binding sites to prevent actin from binding to myosin. Tropomyosin binds to troponin to form a troponin-tropomyosin complex. The troponin-tropomyosin complex prevents the myosin “heads” from binding to the active sites on the actin microfilaments. Troponin also has a binding site for Ca++ ions.
To initiate muscle contraction, tropomyosin has to expose the myosin-binding site on an actin filament to allow cross-bridge formation between the actin and myosin microfilaments. The first step in the process of contraction is for Ca++ to bind to the regulatory protein troponin so that tropomyosin can slide away from the binding sites on the actin strands. This allows the myosin heads to bind to these exposed binding sites and form cross-bridges. The thin filaments are then pulled by the myosin heads to slide past the thick filaments toward the center of the sarcomere. But each head can only pull a very short distance before it has reached its limit and must be “re-cocked” before it can pull again, a step that requires ATP (Fig. 5).
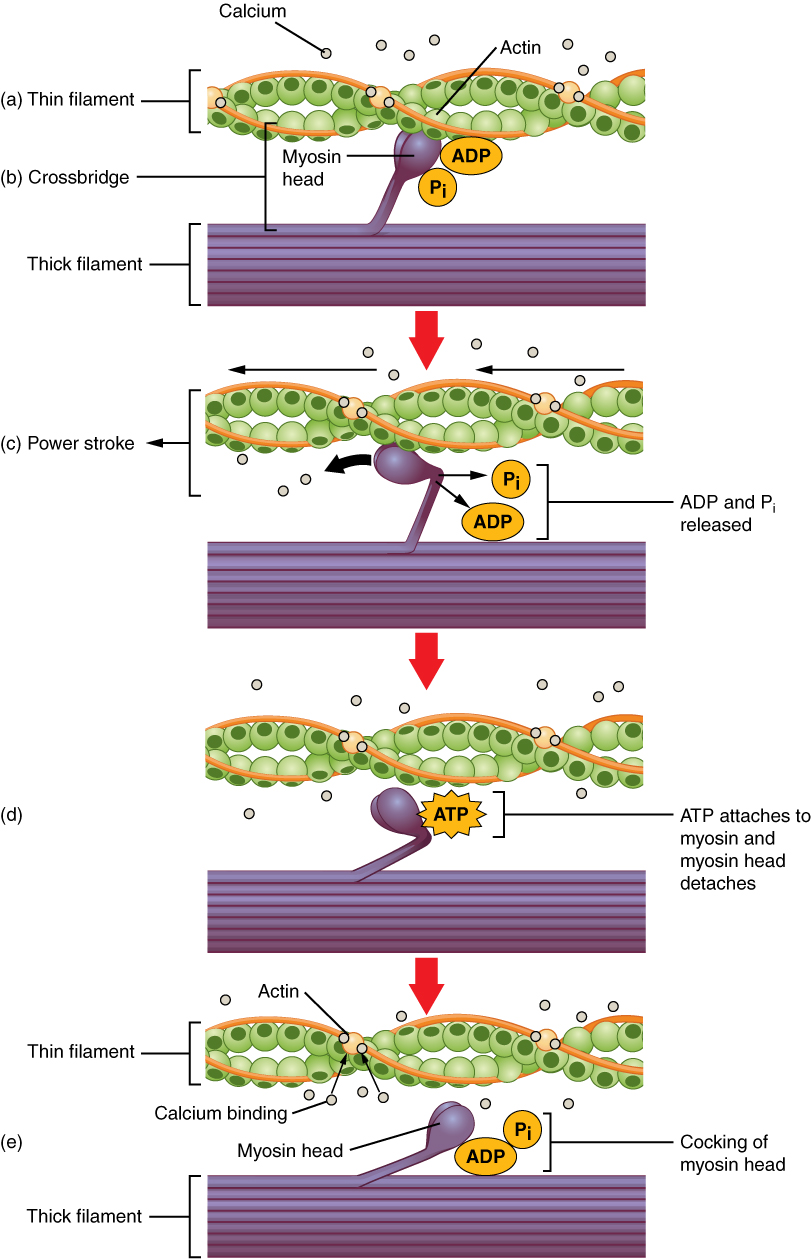
Cross-bridge formation occurs when the myosin head attaches to the actin while adenosine diphosphate (ADP) and inorganic phosphate (Pi) are still bound to myosin (Fig. 5 a,b). Pi is then released, causing myosin to form a stronger attachment to the actin, after which the myosin head moves toward the M-line, pulling the actin along with it. As actin is pulled, the filaments move approximately 10 nm toward the M-line. This movement is called the power stroke, as movement of the thin filament occurs at this step (Fig. 5c). In the absence of ATP, the myosin head will not detach from actin.
One part of the myosin head attaches to the binding site on the actin, but the head has another binding site for ATP. ATP binding causes the myosin head to detach from the actin (Fig. 5d). After this occurs, ATP is converted to ADP and Pi by the intrinsic ATPase activity of myosin. The energy released during ATP hydrolysis changes the angle of the myosin head into a cocked position (Fig. 5e). The myosin head is now in position for further movement.
When the myosin head is cocked, myosin is in a high-energy configuration. This energy is expended as the myosin head moves through the power stroke, and at the end of the power stroke, the myosin head is in a low-energy position. After the power stroke, ADP is released; however, the formed cross-bridge is still in place, and actin and myosin are bound together. As long as ATP is available, it readily attaches to myosin, the cross-bridge cycle can recur, and muscle contraction can continue.
Please cite:
Haen Whitmer, K.M. (2021). A Mixed Course-Based Research Approach to Human Physiology. Ames, IA: Iowa State University Digital Press. https://iastate.pressbooks.pub/curehumanphysiology/
- Section URL: https://openstax.org/books/anatomy-and-physiology/pages/10-3-muscle-fiber-contraction-and-relaxation ↵